Part 3 of: The Decentralized Cybersecurity Paradigm: Rethinking Traditional Models
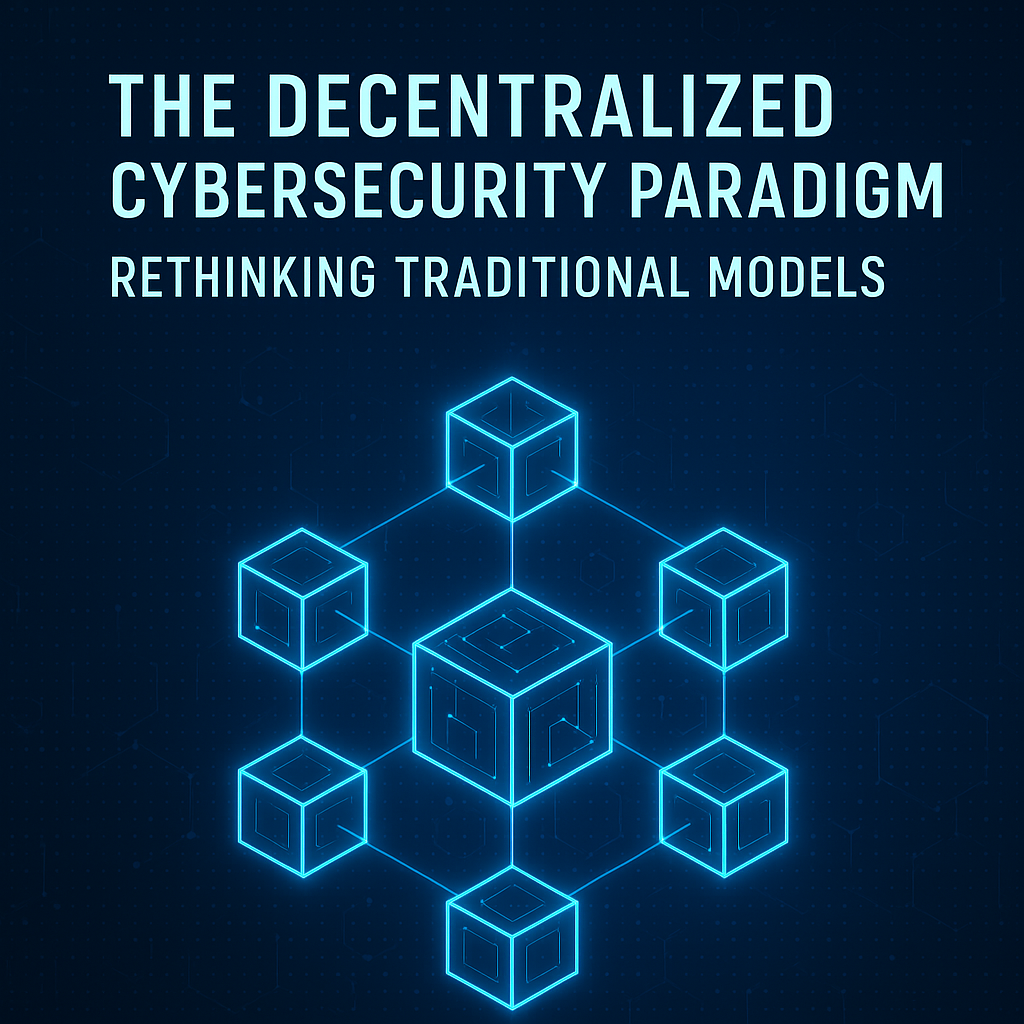
In Part 2 we considered decentralized technology for securing identity data. Now, the time has come to consider the role of zero-knowledge proofs in enhancing data security.
Setting the Stage for Decentralized Cybersecurity and the Promise of Zero-Knowledge Proofs
Without a doubt, traditional, centralized cybersecurity is facing increasing challenges in protecting sensitive data from sophisticated and persistent cyber threats. The continuously expanding attack surface has created numerous vulnerabilities that malicious actors are keen to exploit. A few reasons for this are the rapid adoption of cloud services and the shift towards remote work. Centralized data stores were initially designed to streamline access control. But this has made them prime targets for data breaches due to the vast amounts of sensitive information they store.
This is especially concerning in the identity management space (https://thehackernews.com/2025/03/identity-new-cybersecurity-battleground.html). The compromise of credentials in these systems can grant attackers access to a multitude of resources. In fact, this highlights the limitations of relying on single points of control for security. As cyberattacks grow in sophistication, exploiting weaknesses in these traditional, often fragmented, identity platforms, the need for a paradigm shift in cybersecurity has become increasingly apparent.
As a result, decentralized cybersecurity paradigms have emerged, aiming to distribute control, in turn enhancing resilience against attacks. Among the revolutionary cryptographic tools aligning perfectly with the principles of decentralized security are Zero-Knowledge Proofs (ZKP) (https://csrc.nist.gov/projects/pec/zkproof). ZKPs offer a novel approach to data security by enabling the verification of information without revealing the information itself. This capability establishes trust and maintains security in decentralized environments. However, it does so without the need for central authorities to hold and manage sensitive data. Fundamentally, by moving away from reliance on revealing sensitive data to establish trust, ZKPs offer a foundation that becomes the core of decentralized systems (https://www.chainalysis.com/blog/introduction-to-zero-knowledge-proofs-zkps/).
Demystifying Zero-Knowledge Proofs
Core Principles
At its core, a ZKP is a cryptographic method consisting of two parties, the prover and the verifier. The prover must convince the verifier that a specific statement is true. The catch is, it does so without disclosing any information beyond the mere fact of the statement’s truth. This interaction between prover and verifier follows a defined protocol. The prover demonstrates knowledge of “something” without revealing the “something”itself.The underlying intuition is that it should be possible to obtain assurance about some data without needing to see the actual data or the steps involved for the assurance.
The security value provided by ZKPs relies on three fundamental properties:
- Completeness
- Soundness
- Zero-knowledge
Completeness
Completeness ensures that if the statement being proven is indeed true, an honest prover who follows the protocol correctly will always be able to convince an honest verifier of this fact. This property guarantees that the proof system functions as intended when all parties act honestly.
Soundness
Soundness is a security property that ensures that if the statement being proven is false, no dishonest prover can trick an honest verifier into believing it’s true. This is of course not foolproof and comes with an acceptable probability of error. When successful, this property means that even if a malicious prover deviates from the protocol in an attempt to deceive the verifier, the probability of success is extremely low. Soundness is crucial for the integrity of the proof system, as it prevents the acceptance of false claims as true.
Zero-Knowledge
Zero-knowledge guarantees that the verifier learns nothing from the interaction beyond the fact that some statement is true. Even after successfully verifying the proof, the verifier should not gain any additional information about the prover’s secret or the reason why something is true. This property is very important for privacy-preserving applications, as it ensures that no sensitive information leaks during the proof process.
Example
Let’s resort to the classic cybersecurity characters of Alice and Bob.
The Setup:
- There’s a secure room built into a hill, like a vault with two entrances: DoorA and DoorB.
- Inside the room is a locked interior door that connects the two entrances via a hallway.
- Only someone with the secret key can unlock this interior door to go from one door to the other.
Alice (the Prover) claims to have the key. Bob (the Verifier) wants proof. But Alice refuses to let Bob see the key or watch her use it.
The Protocol (Challenge – Response):
- Alice enters the room through either DoorA or DoorB, chosen at random.
- Bob waits outside the room and doesn’t see which door Alice chooses.
- Once Alice is inside, Bob tells her to “Come out through DoorA” or “Come out through DoorB”
- If Alice has the key, she can:
- Unlock the interior door and exit through whichever door Bob requests.
- If she doesn’t have the key, she can only exit through the door she entered — and must hope Bob picks that one.
- Alice repeats this process multiple times to eliminate the possibility that Bob is just getting lucky when he picks an exit door. If Alice always appears at the door Bob names, he becomes convinced that she truly has the key.
Why is this a Zero-Knowledge Proof?
ZKP Principle | How it’s satisfied in the story |
Completeness | If Alice really has the key, she can always come out the door that Bob calls out. |
Soundness | A fraudulent actor has a 50% chance of guessing correctly each time. Repeating the challenge many times makes fraud statistically unlikely. |
Zero-Knowledge | Bob learns nothing about the key itself or how the interior mechanism works, just that Alice is able to do what only someone with the key could do. |
Some key points:
- The Prover demonstrates something (e.g. possession of a key) via a repeatable challenge–response.
- The Verifier gains confidence while learning nothing that should remain secret.
- No information about the key (the actual proof) is ever disclosed.
Identity Verification Example
Imagine someone asks you to verify your identity online. But, instead of uploading sensitive documents or revealing your exact age, address, or full name, you prove your identity without disclosing a single private detail. That’s the magic of ZKPs.
The Setup:
A secure digital system (e.g. a government portal or online financial service) needs to confirm that you meet a certain requirement (e.g. being over 18 years of age, a verified citizen, etc). But, it should not collect or store your personal data. You, the user, want to prove you meet the requirements without revealing who you are.
The Protocol:
- You (the Prover) hold a verifiable credential issued to you, it is a cryptographic token stating:
- This user is over 18 years of age
- This user holds a valid government ID
- This user has been verified by a trusted issuer
- This user holds a valid government ID
- This user is over 18 years of age
- The Verifier (a website, system, or app) wants assurance that your claim is valid. But they should not learn:
- Your actual birthdate
- Your full name
- Any personal metadata
- Your full name
- Your actual birthdate
- Using a ZKP, your device constructs a cryptographic proof showing the following without revealing the underlying data:
- A valid credential exists
- It was issued by a trusted authority
- It satisfies the policy (e.g. age > 18, etc)
- It was issued by a trusted authority
- A valid credential exists
Just like Alice proves she can walk from one room to another without revealing how, a user can prove they are qualified (e.g. over 18) without showing their exact birthdate. ZKPs allow users to prove only what’s necessary without revealing who they are, creating a privacy-preserving environment.
The Magic of Verification Without Revelation
The core strength of ZKPs lies in their seemingly “magical” ability to enable verification without revelation. This is not just a theoretical concept but a powerful tool with profound implications for building trust and ensuring security in decentralized systems. There are environments where participants don’t inherently trust each other, nor a central authority. ZKPs provide a cryptographic mechanism to establish trust based on mathematical proof rather than reliance on intermediaries who might have access to sensitive data. This capability proves especially valuable in scenarios that require balancing transparency with the critical need for privacy, such as financial transactions, identity verification, and secure data sharing. By allowing for the validation of information or the correctness of computations without exposing the underlying sensitive data, ZKPs pave the way for more secure, private, and trustworthy interactions in an increasingly interconnected and decentralized digital world.
The Power of ZKPs in Enhancing Data Security
Minimizing Data Exposure and Enhancing Privacy
Data security is the goal here. ZKP’s relevant benefit is the ability to minimize data exposure. Traditional methods of proving identity or verifying information often require the disclosure of extensive personal data. For instance, proving one’s age might involve presenting an entire identification document containing much more information than just a date of birth. ZKPs offer a more privacy-centric approach by allowing users to demonstrate that they meet specific criteria without revealing the sensitive data itself. This principle of selective disclosure is a foundational principle of privacy-preserving technologies. It also supports the growing emphasis on data minimization, which multiple regulations (e.g., GDPR) actively promote. By requiring less sensitive information during certain verification processes, ZKPs significantly reduce the risk of data breaches and identity theft.
Building Trust in Decentralized Systems
In trustless environments, such as blockchain networks and other decentralized systems, ZKPs play a crucial role in building an ecosystem of trust. Many environments lack a central authority to vouch for the validity of transactions or data. ZKPs provide a cryptographic mechanism to address this challenge by enabling the verification of transactions, and things like smart contracts, without revealing the underlying sensitive details. For example, in privacy-focused cryptocurrencies, ZKPs are used to create shielded transactions that conceal the sender, receiver, and the amount transacted. This all done while still allowing network participants to cryptographically verify that the transaction is valid and adheres to some set of rules. This capability creates trust among users by ensuring the integrity of the system and the legitimacy of operations without compromising the privacy of the individuals involved.
Different Types of Zero-Knowledge Proofs
Over time the field of ZKPs has seen significant advancements. These developments have led to various practical ZKP schemes, each with its own underlying cryptographic methodologies. The most known ZKPs are:
- zk-SNARKs
- zk-STARKs
- Bulletproofs
Zero-Knowledge Succinct Non-Interactive Arguments of Knowledge (zk-SNARK)
zk-SNARKs rely on advanced cryptographic techniques, primarily Elliptic Curve Cryptography (ECC), to achieve its properties (https://pixelplex.io/blog/zk-snarks-explained/). A key characteristic of zk-SNARKs is their succinctness, meaning they generate proofs that are very small in size, typically just a few hundred bytes. This approach delivers excellent performance, enabling verifications to complete extremely quickly, often within milliseconds, regardless of some statement’s complexity. Furthermore, zk-SNARKs operate in a non-interactive manner, with the prover sending just one message to the verifier to deliver the proof.
However, zk-SNARK schemes often rely on an initial “trusted setup” ceremony. This ceremony involves multiple participants generating cryptographic parameters (proving and verification keys) whose security depends on the secrecy of the entropy used during the setup. If someone compromises this entropy data, they could potentially create fraudulent proofs. Techniques like Multi-Party Computation (MPC) ceremonies help reduce this risk by involving multiple independent parties in the setup process. However, this approach still relies on a trust assumption, which remains a potential limitation. Recent advancements in cryptographic research have led to the development of zk-SNARK schemes that either utilize universal trusted setups (e.g. PLONK) or eliminate the need for them altogether (e.g. Halo).
Despite the trusted setup requirement in some variants, zk-SNARKs have found numerous applications in enhancing data security. Cryptocurrencies like Zcash use zk-SNARKs to enable fully private transactions by hiding the sender, receiver, and transaction amount. Blockchain platforms like Ethereum also apply zk-SNARKs in layer-2 scaling solutions to bundle multiple transactions and verify them off-chain using a single succinct proof. This increases transaction throughput and reduces fees. Beyond these cases, zk-SNARKs are being explored for identity verification systems where privacy is paramount.
Zero-Knowledge Scalable Transparent Arguments of Knowledge (zk-STARK)
zk-STARKs (https://starkware.co/stark/) represent another significant advancement in ZKP technology, specifically designed to address some of the limitations of zk-SNARKs (https://hacken.io/discover/zk-snark-vs-zk-stark/). One of the key differentiators of zk-STARKs is their transparency, as they do not require a trusted setup. Instead, zk-STARKs rely on publicly verifiable randomness and collision-resistant hash functions for their security. This makes this type of system more transparent and eliminates the trust assumptions associated with a setup phase.
Another advantage of zk-STARKs is their scalability, particularly for verifying large and complex computations. The proving and verification times in zk-STARKs scale almost linearly with the size of a computation. This makes for efficient performance. Furthermore, zk-STARKs leverage hash-based cryptography, which has shown great promise in the building of Post-Quantum Cryptography (PQC) algorithms. This possibility positions zk-STARKs as a post-quantum alternative to zk-SNARKs as they often rely on ECC, which is vulnerable to quantum computing advancements.
Despite these benefits, zk-STARKs typically generate larger proof sizes compared to zk-SNARKs. This larger proof size can result in higher verification overhead in terms of computational resources and increased costs when used on blockchain platforms. Nevertheless, the transparency, scalability, and quantum resistance of zk-STARKs make them a promising technology.
Bulletproofs
Bulletproofs represent another significant type of ZKP, particularly known for their efficiency in generating short proofs for statements. Similar to zk-STARKs, Bulletproofs do not require a trusted setup, instead relying on standard cryptographic material, such as the strength of the discrete logarithm problem (https://crypto.stanford.edu/bulletproofs/). This eliminates the trust concerns associated with the setup phase of some zk-SNARKs.
Bulletproofs produce relatively compact proof sizes, generally larger than zk-SNARKs but considerably smaller than zk-STARKs. This introduces an interesting balance between proof size and computational efficiency. A key feature of Bulletproofs is their strong support for proof aggregation (https://www.maya-zk.com/blog/proof-aggregation), allowing multiple proofs to be combined into a single, shorter proof. These become beneficial for transactions with multiple outputs or for proving statements about multiple commitments simultaneously.
While Bulletproofs offer advantages in proof size and the absence of a trusted setup, their verification time scales linearly with the complexity of an underlying compute challenge. Linear scaling can limit performance with very large datasets when compared to the faster verification times achieved by zk-SNARKs or zk-STARKs. Nevertheless, privacy-focused cryptocurrencies like Monero have adopted Bulletproofs for Confidential Transactions to conceal transfer amounts (https://blog.pantherprotocol.io/bulletproofs-in-crypto-an-introduction-to-a-non-interactive-zk-proof/).
The following table summarizes the key differences covered here:
Feature | zk-SNARKs | zk-STARKs | Bulletproofs |
Trusted Setup | Often required | Not required (Transparent) | Not required |
Proof Size | Small (~hundreds of bytes) | Large (~tens of kilobytes) | Compact (~kilobyte) |
Verification Time | Fast (constant or sublinear) | Fast (sublinear to quasilinear) | Linear |
Quantum Resistance | Generally not resistant (relies on ECC) | Resistant (relies on hash functions) | Generally not resistant (relies on discrete log) |
Cryptographic Assumptions | Elliptic Curve Cryptography, pairings | Collision-resistant hash functions | Discrete Logarithm Problem |
Scalability | Scales linearly with computation size | Highly scalable for large computations | Good for range proofs |
Key Applications | Privacy coins, zk-rollups, identity | Scalable dApps, layer-2 solutions | Confidential transactions, range proofs |
Choosing the appropriate type of ZKP depends on the specific requirements and constraints of a data security application. For scenarios where proof size and fast verification are critical, and a trusted setup is acceptable, zk-SNARKs might be the path forward. If transparency and resistance to quantum computing are paramount, and larger proof sizes are tolerable, zk-STARKs would be a consideration. For applications focused on range proofs and confidential transactions, where a trusted setup is undesirable and compact proofs are needed, Bulletproofs offer a compelling option.
Real-World Use Cases of ZKPs in Cybersecurity
ZKPs are not just a theoretical concept; they have found practical applications in various cybersecurity areas, offering innovative solutions to improve both privacy and security.
Private and Secure Authentication Systems
ZKPs have the potential to revolutionize authentication and identity verification systems by enabling passwordless logins and privacy-preserving credential checks. In authentication, users can prove they know their password without transmitting it, eliminating the need for password databases and reducing the risk of data interception or replay attacks. Instead of sending a password to a server, a user’s device generates a ZKP that verifies knowledge of the password without revealing it, significantly enhancing security. Beyond login systems, ZKPs play a crucial role in DID frameworks, allowing users to verify specific credentials without exposing their full digital identity. Selective disclosure allows users to share only the necessary information, preserving privacy while building trust. By enabling verification without revelation, ZKPs reinforce the core principles of Zero-Trust (ZT) security, where systems verify every access request instead of assuming trust.
Privacy-Preserving Data Sharing and Collaboration
ZKPs offer powerful tools for secure, privacy-preserving data sharing and collaboration, especially in contexts involving sensitive information such as medical records or financial data. For example, financial institutions can share aggregated data for fraud detection without exposing individual account details. ZKPs also enable parties to verify the integrity and authenticity of shared data without revealing its actual content. A data holder can prove that a dataset possesses certain statistical properties or that a computation was correctly performed, without disclosing the raw data itself. This capability is critical for building trust and ensuring data quality in collaborative environments where privacy is essential. It allows organizations to extract meaningful insights from sensitive data while maintaining strict confidentiality.
Enabling Anonymous and Secure Transactions
ZKPs are essential for enabling anonymous and secure transactions across a range of applications, particularly in cryptocurrencies. Privacy-focused coins like Zcash use zk-SNARKs to support shielded transactions, encrypting details such as the sender, receiver, and amount on the blockchain while still allowing the network to verify the transaction’s validity under its consensus rules. Likewise, Monero implements Bulletproofs to hide transaction amounts, revealing only the origin and destination. Beyond cryptocurrencies, ZKPs also power secure and anonymous voting systems. In these systems, voters can prove their eligibility and confirm their vote was cast and counted. This would get done without disclosing their identity or vote choice. This preserves individual privacy while ensuring election integrity and transparency. By enabling secure, verifiable, and private interactions, ZKPs effectively address critical privacy concerns in digital environments.
Enhancing the Security of Decentralized Applications (dApps)
ZKPs increasingly enhance the security, privacy, and functionality of decentralized Applications (dApps) built on blockchain platforms (https://www.coinbase.com/learn/crypto-basics/what-are-decentralized-applications-dapps). A key application lies in layer-2 scaling solutions like zk-rollups, which use ZKPs such as zk-SNARKs or zk-STARKs to verify the correctness of computations performed off-chain. These solutions execute transactions and computations away from the main blockchain and submit ZKPs back to the main chain to attest to their validity. The system achieves that without exposing any underlying data. This approach significantly boosts transaction throughput and reduces gas fees while preserving privacy. Additionally, ZKPs enable the development of private smart contracts, allowing sensitive contract terms and execution data to remain confidential. This capability is especially valuable in Decentralized Finance (DeFi), where financial transactions must remain private while still ensuring verifiable execution. By offering a foundation for both scalable and private computation, ZKPs are critical to the growth and innovation of the dApp ecosystem.
Advantages of Leveraging ZKPs for Data Security
Leveraging ZKPs for data security offers an interesting set of advantages that address the evolving challenges of the digital landscape. One of the most significant benefits is the unparalleled privacy and confidentiality they provide by minimizing data exposure. ZKPs inherently limit the amount of information that needs to be shared for verification, ensuring that sensitive data remains hidden during the process. This reduced exposure directly translates to a reduced risk of data breaches and identity theft, as attackers have less sensitive information to target or intercept.
Furthermore, ZKPs enhance trust and transparency in digital interactions. By enabling cryptographic verification without the need for external entities to access the underlying data, they foster a higher degree of trust in decentralized systems and online communications. This trust is built on mathematical proof rather than assumptions or reliance on central authorities.
Challenges of ZKP Adoption
Despite the potential of ZKPs, their widespread adoption is not without challenges.
One of the primary hurdles stems from the computational overhead that ZKPs impose, especially the resource-intensive process of generating proofs. Depending on the complexity of the statement and the specific ZKP scheme in use, the prover often incurs significant computational costs. This can reduce performance and slow down applications, particularly those that rely on real-time verification.
The implementation and integration of ZKPs with existing systems also present considerable challenges. It often requires specialized expertise in cryptography and might necessitate substantial uplift to existing infrastructure. The technical intricacies involved in designing and deploying ZKP-based solutions can be daunting for teams unfamiliar with the underlying mathematical and cryptographic principles.
Scalability can be another concern, particularly for very large-scale applications. While certain ZKP types like zk-STARKs are designed with scalability in mind, the size and verification time of proofs can still become a bottleneck for close to real-time systems that generally have extremely high transaction volumes.
Beyond those challenges, the lack of complete standardization and interoperability across different ZKP schemes and platforms poses a challenge to broader adoption. The variety of ZKP implementations, each with its own specific properties and requirements, can make it difficult to achieve seamless integration and widespread use across diverse systems.
Finally, the “trusted setup” requirement in some popular zk-SNARK schemes introduces a unique challenge related to trust and security. The reliance on a secure and honest generation of the initial cryptographic material is critical. Any compromise during this phase could potentially undermine the integrity of the entire system. While multi-party computation ceremonies aim to mitigate this risk, the inherent need for trust in the setup process remains a point of consideration.
The Future Landscape: Trends and Developments in ZKP Technology for Cybersecurity
Irrespective of the challenges, the field of ZKP technology is rapidly evolving. Many entities see this as a large part of the future of data security. As such, numerous trends and developments are pointing towards an increasingly significant role for ZKPs in the future of cybersecurity overall.
Ongoing research and development are focused on creating more efficient ZKP algorithms and exploring hardware acceleration techniques to improve performance. These advancements aim to make ZKPs more practical and accessible for real-time applications and resource-constrained environments.
Efforts are also underway to develop more user-friendly tools, libraries, and frameworks. The aim here is to abstract away the complexities of ZKP cryptography, making it easier for developers without deep cryptographic expertise to implement and integrate ZKP-based solutions into their systems. This simplification will be crucial for driving broader adoption across various industries.
As the demand for enhanced privacy and security continues to grow, the adoption of ZKPs in diverse cybersecurity applications is expected to increase significantly. This includes wider use in decentralized identity management systems to enable privacy-preserving authentication, in secure authentication protocols to replace vulnerable password-based methods, and in ensuring the confidentiality of transactions in various digital contexts.
The future may also see a greater integration of ZKPs with some Artificial Intelligence fields (https://medium.com/tintinland/advantages-and-challenges-of-zero-knowledge-machine-learning-4625f5bb2053) as well as other privacy-enhancing technologies, such as homomorphic encryption and secure multi-party computation.
Given the potential threat posed by quantum computing to current cryptographic algorithms, research into quantum-resistant ZKP schemes is gaining momentum (https://upcommons.upc.edu/bitstream/handle/2117/424269/Quantum_Security_of_Zero_Knowledge_Protocols.pdf). Developing ZKP protocols that rely on cryptographic primitives known to be resistant to quantum attacks will be essential for ensuring the long-term security of ZKP-based systems.
Finally, there are ongoing standardization efforts aimed at promoting interoperability and establishing common protocols and frameworks for ZKPs (https://cryptoslate.com/standards-for-zero-knowledge-proofs-will-matter-in-2025/). Standardization will be crucial for facilitating the seamless integration of ZKPs across different platforms and applications, paving the way for their widespread adoption and use in enhancing cybersecurity.
ZKPs: Rethinking Data Security in the Decentralized Era
ZKPs stand at the forefront of a transformative shift in how we approach data security. This is, particularly within the emerging context of decentralized cybersecurity. By enabling the verification of information without revealing the sensitive data itself, ZKPs offer a powerful cryptographic tool that addresses the inherent limitations of traditional, centralized security models. Their ability to minimize data exposure, enhance privacy, and build trust in decentralized environments positions them as a solid technology for the future of secure digital interactions.
As things move forward in an increasingly interconnected world where data breaches and privacy concerns are ever-present, the potential of ZKPs to revolutionize how we conduct secure transactions is immense. While challenges related to computational overhead, implementation complexity, and standardization remain, the ongoing advancements in ZKP research and development are steadily addressing these limitations.
In conclusion, ZKPs represent a fundamental rethinking of data security in the decentralized era. By embracing the principle of “verify without revealing,” ZKPs empower individuals and organizations to engage in the digital world with greater confidence, knowing that their sensitive information can be protected while still enabling secure and trustworthy interactions. As this technology continues to mature and find broader adoption, it holds the key to unlocking a more private, secure, and resilient digital future for all; hence, we have explored the role of zero-knowledge proofs in enhancing data security.
Part 4 of this series aims to cover decentralized security system resilience.